A puzzle piece from stellar chemistry could change our measurements of cosmic expansion
Astronomers led by Maria Bergemann (Max-Planck-Institute for Astronomy) have performed chemical measurements on stars that could markedly change the way cosmologists measure the Hubble constant and determine the amount of so-called dark energy in our universe. Using improved models of how the presence of chemical elements affects a star’s spectrum, the researchers found that so-called supernovae Type Ia have different properties than previously thought. Based on assumption about their brightness, cosmologists have used those supernovae to measure the expansion history of the universe. In light of the new results, it is now likely those assumptions will need to be revised.
Over the past decade or so, when Maria Bergemann, a Lise Meitner Research Group leader at the Max Planck Institute for Astronomy, developed improved ways of measuring the chemical properties of stars, she didn’t know that her research might one day affect the way that astronomers measure cosmic expansion, the Hubble constant, and the amount of Dark Energy in our universe. But via a series of unforeseen connections, that is what appears to be happening right now. Using the analysis tools Bergemann developed, the astronomers were able to trace the abundance of the chemical elements manganese and iron over the past 13 billion years of galactic history. Their (unexpected) result puts constraints on the properties of the stellar explosions, so-called Supernovae of Type Ia, which are needed to produce the element.
Previously, it was believed that the majority of type Ia supernovae were caused by a White Dwarf star orbiting an ordinary star, sucking off the hydrogen from the star’s outer layers. The manganese abundances of stars within our Milky Way show that, on the contrary, three in four such explosions result from other kinds of type Ia supernovae. One of them could be a scenario in which two White Dwarf stars orbit each other. The other scenario involves a white dwarf, which accretes helium from a companion and experiences sequential "outside-in" detonations. The difference between the standard scenario and alternative explosion mechanisms for SN Ia may have fundamental consequences for the relation between the brightness maximum, the way the brightness changes over time, and the overall time scale of these supernova explosions. That, in turn, is important for some of the most basic observations of cosmology. Those observations use supernovae of Type Ia as “standard candles,” that is, as light sources whose intrinsic brightness can be determined from observations. Comparing a source’s intrinsic brightness and its observed brightness allows astronomers to determine the source’s distance to us. Detections of the so-called Dark Energy thought to be responsible for around 70% of our universe’s total energy density go back to observations of this kind, as do measurements of the Hubble constant that specifies the current expansion rate of our universe. If the supernovae used for those measurements are not standard candles of one of the same type, but instead are of at least two different types whose intrinsic properties are systematically different, the cosmological deductions will need to be revisited.
Modelling stellar spectra as never before
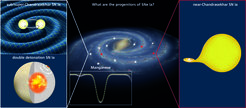
Bergemann’s analysis tools are the latest in a series of developments that can be traced back to Robert Bunsen and Gustav Kirchhoff, who invented spectroscopy as a chemical tool in Heidelberg in the second half of the 19thcentury. They found that certain narrow bright or dark regions in a spectrum, spectral lines, could be traced to the presence of specific chemical elements. By the early 20thcentury, astronomers were using simplified models for those spectral lines to examine the atmospheres of stars – leading to the measurement of stars’ temperatures, surface pressures, and chemical composition. But those models assumed that stars were perfect spheres (in contrast with the three-dimensional complex structure of real stars) and that their pressure and gravitational force were in equilibrium (known as hydrostatic equilibrium). The models also assumed, at least locally, “thermodynamic equilibrium” between gas and radiation – i.e. in each small region, the available energy had had time to spread evenly between the different parts of the system, allowing us to assign one temperature to each region.
Models of stellar atmospheres, and of the radiation emitted by stars, that do not assume local thermodynamic equilibrium are a relatively recent development. Such novel models are known as Non-LTE models (since they assume No Local Thermal Equilibrium). These models go hand in hand with three-dimensional simulations of the convection beneath the star’s surface, similar to the motion of boiling water in a pot, with matter moving upwards in some regions, downwards in others. They also include the interaction of highly-dynamic plasma with the star’s radiation. The theory behind Non-LTE and hydrodynamics had already been formulated in the late 1970s. However, applying these models to the analysis of chemical composition of many stars in the Galaxy only became possible about 20 years ago. This was when powerful modern supercomputers became available for scientific research, coinciding with recent advances in the description of atomic structure and light-matter interactions that resulted in robust data for atomic physics, which is needed in Non-LTE models. Bergemann has worked on different aspects of such models since 2005, making her one of the pioneers of the field.
For some elements, notably iron, the new, refined methods yield virtually the same result as their simplified precursors. But for others, there are notable differences. Bergemann and her team, including Andrew Gallagher, Camilla Juul Hansen, and Philipp Eitner found an example of this when tracing the chemical evolution of the element manganese, a metal next to iron in the periodic table of elements. Gallagher succeeded to greatly improve the performance of 3D Non-LTE code. Hansen delivered high-quality observational data covering the spectral regions essential for the observations, which are in the near-ultraviolet. Eitner, as a bachelor student at the University of Heidelberg, worked out a robust framework to apply Non-LTE to the modelling of stellar spectra. He also extended that analysis to cases where we can observe a spectrum not for separate stars, but only for the combined light from numerous stars in a stellar cluster. This is essential for the analysis of extragalactic star clusters.
Tracing the history of manganese in our galaxy and beyond
By analyzing 42 stars, the astronomers were able to reconstruct the history of manganese production within our galaxy. Chemistry-wise, the universe started very simple, with almost nothing else but hydrogen and helium shortly after the Big Bang, 13.8 billion years ago. A large fraction of heavier elements have been produced between now and then in the interior of stars. Other elements – like manganese and iron – are produced in the violent supernova explosions marking the end of certain stars’ lives. Supernovae disperse the exploding star’s matter, seeding the surrounding space with heavier elements. As stars of later generations form, they will incorporate those heavier elements. Spectral traces of these elements will be observable in the stars’ atmospheres. (Incidentally, the heavier elements in the swirling gas disk around the newborn star are the chemical basis for the formation of planets and, in the case of our Solar System, ultimately for the formation of life on one of those planets. Without those elements, we would not exist, and neither would the Earth.)
Because of this kind of cumulative chemical history, the abundance of elements such as iron in a star’s atmosphere is a direct indicator of how long ago that star was born. Using high-resolution stellar spectra from 8-10 meter telescopes – both ESO’s Very Large Telescope and the Keck Observatory – Bergemann and her colleagues measured the abundances for both iron and manganese for 42 stars, some as old as 13 billion years. Using the iron abundance as an indicator of each star’s age relative to the others, the astronomers were able to reconstruct the history of manganese production in our galaxy. To their considerable surprise, the new and improved analysis showed that the ratio of manganese to iron was fairly constant over that long period. Earlier, less refined studies had found a trend in the manganese production steadily increasing over the past 13 billion years of Galactic history. Even more surprisingly, the astronomers found the same constant ratio between manganese and iron in all the different regions of our own Galaxy, and also in nearby galaxies of the Local group. At least in our cosmic neighbourhood, the manganese/iron ratio appears to be a universal chemical constant.
Supernovae with a fundamental limit
This is where the supernovae come into play. Manganese needs the impressively high energy liberated in supernova explosions to form. Different types of supernovae produce iron and manganese in different ratios. One of the contributors are so-called core collapse supernovae, where a massive star collapses at the end of its life, having exhausted its nuclear fuel in the core. The others are more interesting in this context: If a White Dwarf star, a remnant of a star similar to the Sun, is orbiting a giant star, its gravity will pull hydrogen from the giant star onto its own surface. Once a limiting mass is reached, the so-called Chandrasekhar mass, the White Dwarf becomes unstable, resulting in a thermonuclear explosion, a so-called Supernova of Type Ia. The limiting mass follows from the fundamental principles of physics, as was first discovered by Subrahmanian Chandrasekhar in 1930. Given the fundamental “Chandrasekhar limit,” in this scenario the total mass of the exploding star, and thus the total brightness of the explosion, is always about the same.
This is good news for astronomers tracing the expansion of our cosmos: When they observe such an explosion, they knowhow bright it is, at the source; by comparing this brightness with the observed brightness, they can deduce the supernova’s distance. Measuring the redshift of the galaxy where the explosion took place (i.e. how fast the galaxy in which the explosion happened is moving away from us), cosmologists can write down a redshift-distance relation, which tells them how fast our universe is expanding (encoded in the so-called Hubble constant) and also whether that expansion is accelerating or becoming slower over time. The late 1990s discovery that our universe is accelerating resulted in the 2011 Nobel Prize in Physics for Saul Perlmutter, Brian Schmidt, and Adam Riess. The acceleration can be explained by assuming that our cosmos is filled with an unusual ingredient that astronomers call 'Dark Energy'.
A different take on supernovae Ia
That, at least, is the story so far. With the previous, less accurate manganese measurements, astronomers had concluded that a significant fraction of Supernovae Type Ia happens in the way described above, with a White Dwarf gobbling up hydrogen from a giant companion star. But in order to explain why the manganese-to-iron ratio has been constant throughout the Galactic history, things must have been different. There are several other ways of producing a Supernova of Type Ia. To observers measuring the explosion’s light curve, that is, the way that its brightness changes over time, these scenarios are indistinguishable from the White-Dwarf-plus-giant scenario.
In one special case, a star accretes matter from a companion that leads to nuclear instability in the outer helium shell, triggering an off-center explosion and a detonation front. This burning front propagates into the core of the star at supersonic speed, triggering another detonation in the carbon-oxygen core of the White Dwarf. This scenario is known as double-detonation SN Ia.
In the other case, the protagonists are two White Dwarf stars in narrow orbit around each other. By the time that the stars have become so close that, in effect, their outer gas swirls around them as a common envelope, gravitational waves emitted by the orbiting binary force the White Dwarfs ever closer together. As the two White Dwarfs merge, the result is a thermonuclear explosion.
Last but not least, even double White Dwarf binaries can experience a double-detonation, resulting into a "dynamically driven double-degenerate double-detonation" SN Ia.
In all these alternative scenarios, the brightness of that explosion is not fixed by a physical constant. The double-detonation explosions do not require the star to attains a Chandrasekhar mass limit: in fact, they explode at lower masses and are, therefore, called sub-Chandrasekhar explosions. In a violent merger, the combined exploding object may be less or more massive than the Chandrasekhar limit. Sub-Chandrasekhar mass explosions are bound to be a bit less bright, while super-Chandrasekhar explosions are expected to be brighter than their Chandrasekhar-mass kin. That is bad news for cosmologists who rely on Supernova Ia standard candles, where such explosions should have a uniform, well-defined intrinsic brightness. What is even worse is that, in order to explain the observed constant ratio of manganese to iron, Bergemann and her colleagues needed to assume that three quarters of all Supernova Ia explosions in our Galaxy are due to compact binary White Dwarf explosions or due to double-detonation explosions. Non-standard Supernovae Ia are the rule, not the exception.
Next steps
This is where the matter stands. There is no doubt that other groups will put the results by Bergemann and her colleagues to the test. Even now, there is corroborating data: A group of astronomers around Evan Kirby and Mia de los Reyes at the California Institute of Technology has found similar results for a number of dwarf galaxies.
The next data release (DR3) of ESA’s Gaia satellite, due in 2021, could yield additional data about the prevalence of double White Dwarf binaries, potentially bolstering the case for the new type of Supernova Ia. Much later, the spaceborne gravitational wave detector LISA, currently scheduled for launch in 2034, will be able to detect the gravitational wave mergers of White Dwarf binaries to great distances, allowing for a direct check of the predictions by Bergemann and her colleagues.
In the meantime, the cosmologists will be busy checking up on what consequences the new Supernova type might have for their deductions about the universe as a whole. In one respect, the expected corrections might even be welcome: Currently, there is a discrepancy about the Hubble constant as measured by using Supernovae of Type Ia and the Hubble constant as measured using left-over radiation (“Cosmic Microwave Background”) from the earliest phases of our cosmos. The new results on Supernovae Ia might help us make the current cosmological models and observations more consistent. All in all, an impressive demonstration of the interconnectedness of astronomical research. Develop a new method of analyzing the chemistry of the stars, and you might just end up effecting a change of our view of the universe as a whole.
Background information
The results described here are documented in P. Eitner et al., “Observational constraints on the origin of the elements: III. Evidence for the dominant role of sub-Chandrasekhar SN Ia in the chemical evolution of Mn and Fe in the Galaxy,“accepted for publication in the journal Astronomy & Astrophysics.
The 3D Non-LTE modelling techniques for Mn are described in Bergemann et al. 2019 "Observational constraints on the origin of the elements. I. 3D NLTE formation of Mn lines in late-type stars", Astronomy & Astrophysics, Volume 631, id.A80, and in Gallagher et al. 2019 "Observational constraints on the origin of the elements. II. 3D non-LTE formation of Ba II lines in the solar atmosphere", Astronomy & Astrophysics, Volume 634, id.A55.
The MPIA researchers involved are Maria Bergemann, Philipp Eitner, Andy Gallagher, and Camilla Juul Hansen in collaboration with Manuel Bautista (Western Michigan University), Andrey Belyaev (Herzen University), Mats Carlsson (University of Oslo), Gabriele Cescutti (INAF and IFPU, Trieste), Remo Collet (Aarhus University), Søren Larsen (Radboud University), Jorrit Leenaarts (Stockholm University), Anja Mayriedl (Montessori-Schule Dachau), Bertrand Plez (Université de Montpellier), Ivo Seitenzahl (University of New South Wales) and Svetlana Yakovleva (Herzen University).
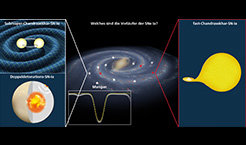