News from the Initiative
Prebiotic Vitamin B3 Synthesis in Carbonaceous Planetesimals
Aqueous chemistry within carbonaceous planetesimals is promising for synthesizing prebiotic organic matter essential to all life. Meteorites derived from these planetesimals delivered these life building blocks to the early Earth, potentially facilitating the origins of life. Here, we studied the formation of vitamin B3 as it is an important precursor of the coenzyme NAD(P)(H), which is essential for the metabolism of all life as we know it. The study of the HIFOL collaboration made it to the cover of the interdisciplinary journal ChemPlusChem.
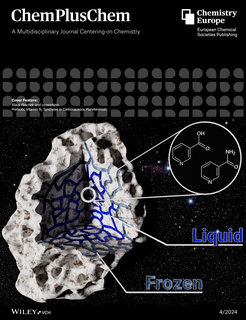
The corresponding study looked at the formation of the vitamin B3 in space. In the nascent solar system, the first larger rocky bodies were formed. These early asteroids, also called planetesimals, were very porous, and the decay of radioactive elements heated the core region of these bodies. This allows water ice trapped in these pores during the formation process to melt and remain liquid for up to several hundred million years. The water contains many simple molecules that have already formed in the depths of space. By meeting and reacting with each other in the liquid water, they can form more complex organic molecules.
One of these is vitamin B3, an essential building block of all life as we know it. The planetesimals were broken up by collisions with each other, and fragments fell to Earth as meteorites. Here they might have helped to set the stage for the origins of life on our planet. Even today, meteorites are recovered and studied in the laboratory, and the presence of vitamin B3 in this material from space has been clearly detected and confirmed. The Japanese space agency JAXA sent the spacecraft Hayabusa2 to the carbon-rich asteroid Ryugu and brought samples back to Earth for laboratory studies, also detecting vitamin B3. Ryugu might be very similar in composition to the planetesimals, from which the meteorites that seeded the early Earth with vitamin B3 originated. Vitamin B3 is present in all life and our bodies in the form of the coenzyme NAD(P)H. Without this coenzyme, there would be no metabolism possible and no organism on Earth could supply itself with energy and survive.
With this work and journal cover, the authors Klaus Paschek, Mijin Lee, Dmitry Semenov, and Thomas Henning hope to encourage the scientific and public community to focus on the crucial role of vitamin B3 in the origins of life, its formation already in space, and therefore its potential onmipresence in the Universe.
Related publication:
From Space to Earth: Uncovering the Potential Role of Meteorites in Kick-Starting Life
A collaboration of HIFOL scientists from the Max Planck Institute for Astronomy, McMaster University, the Institute for Theoretical Astrophysics at Heidelberg University, and Ludwig Maximilian University of Munich has extended our understanding of how the building blocks of life might have reached our planet and helped to give birth to the first living organisms.
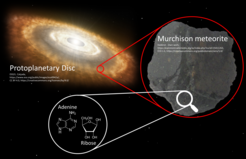
Many of these necessary life-building blocks, so-called prebiotic molecules, were discovered in carbonaceous chondrites — a specific class of meteorites. These molecules could have played a crucial role in the formation of the first RNA molecules on early Earth. RNA molecules are believed to be a critical intermediate step toward the emergence of living systems. RNA can store genetic information, catalyze its own polymerization, and self-replicate, crucial functions associated with all life. This solves an old “chicken-or-the-egg” dilemma in the origins of life, as modern life needs many mutually dependent and complex molecules to achieve these functions. But RNA alone can impersonate all these roles, and might therefore be the stepping stone between dead and alive matter. Later in evolution, DNA might have taken over from RNA and now stores the genetic blueprints of all modern life, including us.
However, the origins of life on Earth are still a mystery and an ongoing topic of research. To explain the emergence of the first RNA, two main scenarios have been proposed: freshwater ponds or hydrothermal vents. Nevertheless, the concentration of nitrogen necessary for nucleobase synthesis in hydrothermal vents is unlikely to be reached, making the exogenous delivery of life-building blocks from space to ponds a potential necessity. In freshwater basins or ponds, wet-dry cycles promote the polymerization and formation of oligonucleotide chains, the first snippets of RNA that might grow larger and larger.
The authors Klaus Paschek, Dmitry Semenov, Ben Pearce, Kevin Lange, Kai Kohler, Thomas Henning, Ralph Pudritz, and Oliver Trapp investigated the formation of nucleobases — the letters of the genetic code in RNA and DNA — inside parent body planetesimals of carbonaceous chondrites. In two published studies, they found that the synthesis of prebiotic organic matter in meteorites could be explained by a combination of several factors: (1) radiogenic heating, (2) aqueous chemistry, and (3) a reduced initial volatile content in the inner regions of the early solar system.
To understand the initial content of volatiles, the source material for the nucleobase synthesis in meteorites, measurements of their behavior at cold temperatures prevailing in the early solar system were provided to the study by Jiao He, head of the Origins Lab at the Max Planck Institute for Astronomy.
In addition, ribose, another key building block of RNA, has been found in meteorites. Using laboratory experiments conducted in the chemistry lab of Oliver Trapp at the Ludwig Maximilian University of Munich, the authors concluded that ribose might form through the formose reaction inside carbonaceous chondrite parent bodies.
These findings suggest that life-building blocks of the RNA world could have been synthesized inside parent bodies and later delivered to the early Earth. Meteorites continue to seed our planet with these organics until the present day, and could have played a crucial role on the early Earth. The search for the origins of life on Earth is ongoing, and these new findings shed light on the possible role of meteorite impacts as the kick-starter for the emergence of life on our home planet and beyond.
Related publications:
2017 Cozzarelli Prize
Physical and Mathematical Sciences
Origin of the RNA world: The fate of nucleobases in warm little ponds
Ben K. D. Pearce, Ralph E. Pudritz, Dmitry A. Semenov, and Thomas K. Henning
A commentary by David Deamer is available.
Listen to an interview with Ben K. D. Pearce
MPIA scientist awarded renowned Cozzarelli Prize
During the weekend, the National Academy of Sciences of the USA presented this year's Cozzarelli Prize in a festive ceremony. Together with their Canadian colleagues Ben Pearce and Ralph Pudritz, Dimitry Semenov and Thomas Henning from the Max Planck Institute for Astronomy (MPIA) were awarded for one of their outstanding publications to explore the origins of life, which appeared in the PNAS Journal in 2017.
Since 2005, the National Academy of Sciences of the United States of America has awarded the Cozzarelli Prize. Yearly, the academy honours research publications of outstanding scientific excellence and originality published the year before in the renowned PNAS Journal (Proceedings of the National Academy of Sciences). PNAS exists since 1915 and covers a broad range of cutting-edge research in the fields of biology, physics and social sciences. Since 2007, the award has been named after the well-known American biochemist Nicholas Robert Cozzarelli (1938-2006), who was editor-in-chief between 1995 and 2006. The prize is awarded annually by the editors. The selection is based on all publications from the previous year.
Last Sunday (29.04.2018), Ben Pearce, Ralph Pudritz, Dimitry Semenov and Thomas Henning were awarded the Cozzarelli Prize for 2017 at the PNAS Editorial Board Meeting in Washington DC in a ceremony for their publication Origin of the RNA world: The fate of nucleobases in warm little ponds (PNAS Oct 2017, 114 (43) 11327-11332).
For many decades it has been a hot research topic to explore how the first building blocks of life - i.e. substances from organic chemistry - appeared on earth about four billion years ago. How could so-called nucleobases such as Adenine, which formed the basis for the first life on Earth, be enriched?
In fact, the publication of the Canadian and Heidelberg authors can be described as outstanding and of originality, because the authors have chosen an extremely interesting and currently still rather unusual approach to investigate this question.
They chose carbon-containing meteorites as the source of the original building blocks and modeled the life-span of the nucleobases in small warm freshwater ponds on volcanic land masses, in which biological evolution could then start.
With this approach, however, the authors argue against the usual way of thinking, the paradigm of biologists, namely that life was created in the oceans and the original building blocks evolved due to the triggering by chemical energy.
However, this approach may seems obvious to modern astronomers, since astrophysicists have already found complex organic molecules even in extremely cold clouds of gas and dust in the Milky Way and also in other hostile to life environments in space. And in particular in meteorites and comets numerous organic substances have been found, making such objects to interesting candidates for possible sources of the original building blocks of life, because our planet was exposed to a bombardment from these celestial bodies in its early days.
The work of Pearce, Pudritz, Semenov and Henning documents the remarkable rise of this reserach area and of astrochemistry to an extremely modern, forward-looking field of research over the last 15 years in which interdisciplinarity plays an important role. With the HIFOL initiative (Heidelberg Initiative for the Origins of Life, see http://www.mpia.de/HIFOL) launched by Thomas Henning and Oliver Trapp, MPIA has been trying for several years to bring scientists together for the research in this field.
Further infomation related to the publication:
http://www.mpia.de/news/science/2017-10-rna-ponds
Bringing the building blocks of life down to Earth, from space
Astronomers from McMaster University and the Max Planck Institute for Astronomy have completed calculations that lead to a consistent scenario for the emergence of life on Earth, based on astronomical, geological, chemical and biological models. In this scenario, life forms a mere few hundred million years after Earth’s surface was cool enough for liquid water; the essential building blocks for life were formed in space during the formation of the solar system, and delivered to warm little ponds on Earth by meteorites. The new results have been published in the Proceedings of the US National Academy of Sciences.
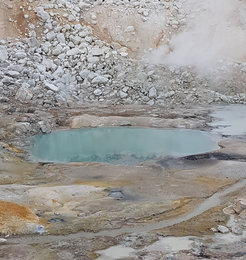
How life began on Earth, roughly 4 billion years ago, is one of the great scientific questions. New results from scientists at McMaster University and the Max Planck Institute for Astronomy suggest a key role for meteorites landing in warm little ponds, delivering essential organic molecules that kick-started the emergence of life in the shape of self-replicating RNA molecules.
The astronomers reached their conclusions after assembling models about planet formation, geology, chemistry and biology into a coherent quantitative model for the emergence of life. Perhaps the most interesting result from these calculations is that life must have emerged fairly early, while Earth was still taking shape, only a few hundred million years after the Earth had cooled sufficiently to allow liquid surface water, such as ponds or oceans. The building blocks of life would have been brought to Earth by meteorites during an era when Earth’s bombardment by such small extraterrestrial rocks was much more intense than today.
Lead author Ben Pearce (McMaster University) says: “No one’s actually run the calculation before. It’s pretty exciting.” His colleague and co-author Ralph Pudritz adds, “Because there are so many inputs from so many different fields, it’s kind of amazing that it all hangs together. Each step led very naturally to the next. To have them all lead to a clear picture in the end is saying there’s something right about this.”
Thomas Henning from the Max Planck Institute for Astronomy, another co-author, says: “In order to understand the origin of life, we need to understand Earth as it was billions of years ago. As our study shows, astronomy provides a vital part of the answer. The details of how our solar system formed have direct consequences for the origin of life on Earth.”
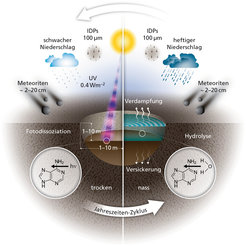
The new work supports the “warm little pond” hypotheses for the origin of life, with RNA polymers forming in shallow ponds, during cycles in which the pond water evaporates and is refilled periodically. It shows how meteorites could have transported a sufficient amount of nucleobases to thousands of such ponds on Earth, helping to kick-start the emergence of self-replicating RNA molecules in at least one of those ponds.
Dmitry Semenov of the Max Planck Institute for Astronomy adds: “Based on what we know about planet formation and the chemistry of the solar system, we have proposed a consistent scenario for the emergence of life on Earth. We have provided plausible physical and chemical information about the conditions under which life could have originated. Now it’s the experimentalists turn to find out how life could indeed have emerged under these very specific early conditions.”
Background information
The work described here has been published as B. K. D. Pearce et al., “Origin of the RNA World: The Fate of Nucleobases in Warm Little Ponds” in the Proceedings of the National Academy of the United States of America.
The article text is available under embargo from the PNAS website.
The co-authors are Ben K. D. Pearce (McMaster University), Ralph E. Pudritz (McMaster University, Max Planck Institute for Astronomy and Center for Astronomy Heidelberg), Dmitry Semenov and Thomas K. Henning (both Max Planck Institute for Astronomy).
In-depth description:
The origin of life on Earth is one of the fundamental questions of science. While we are far from a definite answer, several interesting possibilities have emerged over the past decades. One, worked out in more detail in the 1980s, is the role of an "RNA world". The genetic information of higher organisms is stored in the double helix of DNA molecules. But there are closely related molecules, RNA (ribonucleic acid), which play a prominent role in modern cells; notably, they catalyze certain chemical reactions, and are essential for ferrying genetic information around inside the cell, and for the cell to synthesize specific proteins (the "executive orders" of cell government) based on genetic information. For some viruses, storing genetic information does not involve DNA at all; instead, all the information is encoded in virus RNA.
DNA and RNA
The key building blocks of both RNA and DNA are the nucleotides – in both cases, the pattern within the long, long chain of nucleotides determines the information carried by DNA and RNA. For DNA, this is sometimes expressed as a string where each character stands for one of the four possible nucleobases that form the key part of each DNA nucleotide, A for adenine, C for cytosine, T for thymine, and G for guanine (CGATTCACGATTACA...). In RNA molecules, thymine is replaced by Uracil, U. Another difference: While DNA is commonly found as the well-known double-stranded helix, RNA is more versatile in appearance – most common are single strands of RNA, folded in on themselves in what sometimes can become rather complicated shapes.
While RNA is essential for life as we know it, it also has several key properties that make it a good candidate for earlier, more primitive forms of life – before the advent of cells, to say nothing about multicellular organisms. The most important of these is the property to self-replicate – a given piece of RNA can gather the right nucleotides and arrange them into a copy of itself.
An early RNA world
The most promising current scenario for the emergence of life involves the formation of chains of nucleotides in the shape of RNA, self-replicating the emergence of simple cell precursors as fatty acids spontaneously self-assembled into membranes (a reaction that has been observed in the lab), forming primitive bag-like enclosures which allowed more complex chemical reactions to take place in their protected interior. From these simple beginnings, more complex mechanisms evolved, in particular those of DNA replication.
All the transitions of this scenario are, at this time, speculative, and for each step, alternative explanations and models exist – even for the notion of an RNA world preceding the DNA world, there are alternatives. But we live in exciting times, and there is a realistic hope that the next few decades will see standard model for the origin of life established. Progress will require not just imaginative scenarios, but concrete calculations and experiments, showing which evolutionary pathways are feasible and which aren't. Advances involve different areas of research: On the one hand, more and more hypotheses about the transition from pre-life to life have become amenable to experimental tests, as our knowledge of molecular biology increases. On the other hand, there are exciting new developments at the interface of molecular biology and astronomy.
Over the past few decades, astronomers have made considerable progress in understanding how planetary systems form around young stars, and in particular about the evolution history of the Earth and our own solar system. The new results profit from the wave of exoplanet discoveries, and from direct observations of young planetary systems that have only become feasible with the advent of the most recent generation of telescopes. Planet formation models, including models of the evolving chemistry of newborn planetary systems, set the scene for the conditions under which life could have emerged four billion years ago in our own solar system, and how it could have formed in other planetary systems.
Combining astronomy, chemistry and biology
Now, a study by the astronomers and planet formation specialists Ben Pearce, Ralph Pudritz, Dmitry Semenov and Thomas Henning is drawing together astronomy and prebiotic chemistry to shed light on the earliest era of the RNA world: the processes by which short RNA molecules band together to form longer molecules ("polymerization"), which then start to self-replicate in earnest in a later phase of chemical evolution.
Making longer RNA molecules is not easy, and requires certain well-defined conditions. One possible scenario locates the first steps towards life in the vicinity of hydrothermal vents in the deep ocean – fissures in the Earth's crust emitting water heated by the Earth's deeper, hotter layers. But there are questions about how longer polymers could form under these conditions; polymerization would seem to require a cycle of wet and dry conditions, unlikely to occur deep in the ocean. There is also the problem of obtaining a suitable supply of nitrogen in the shapes of molecules like hydrogen cyanide (HCN) or ammonia, necessary for forming the first stages of life as we know it.
The appeal of warm little ponds
An attractive alternative as a likely birthplace of like are "warm little ponds": small, stagnant bodies of water, in which chemicals can concentrate, and react, under much more favorable conditions than in the ocean. Ponds with walls made of clay or other minerals create particularly favorable conditions, which would foster certain kinds of chemical reaction. An important feature of such ponds is the existence of wet-dry cycles. Every so often, such a pond would dry out, concentrating its chemical content even more and allowing bonding to occur between nucleotides. At some later date, the pond would re-fill with water. Such cycles very probably played a role in shaping the chemical reactions in such ponds. The phrase "warm little pond" itself goes back to one of the earliest speculations on the origin of life: an 1871 letter from Charles Darwin to the botanist Joseph Hooker.
Warm little ponds would have been comparatively rare four billion years ago, when oceans dominated Earth's surface even more than they do today, and when continents were just starting to rise, consisting mostly of igneous rocks created from the mantle, such as basalts. Violent volcanic eruptions were commonplace, and the atmosphere was dominated almost completely by volcanic gases. So were could the organic molecules have come from that set off the evolution of the RNA world?
Building blocks from outer space
A plausible answer, perhaps surprisingly, is that the building blocks for emergent life on Earth or similar planets could have come directly from outer space. The disks, made of gas and dust, that surround young stars contain considerable amounts of ammonia (NH3) and hydrogen cyanide (HCN), both of which providing the nitrogen necessary for forming nucleobases. Iced-over dust particles in all but the innermost regions of such disks turn out to be surprisingly effective little chemical laboratories – in fact, experiments in a laboratory setting here on Earth show how molecules collecting on the icy surfaces of such dust grains can be processed into nucleobases when the grains are illuminated by UV light, such as would be the case for young stars.
In these experiments, scientists were able to show how three of the five nucleobases (namely uracil, cytosine, and thymine) formed spontaneously under such conditions. Meteorites are observed to contain considerable amounts of three of the five nucleobases (namely guanine, adenine, and uracil). It has been shown that these nucleobases are synthesized in the interiors of these meteorite’s “parent bodies,” namely large asteroids, during the formation of the solar system.
Meteorites and dust particles as a cosmic delivery service
Back to the warm little ponds – ideal environments for RNA molecules to become more complex, but where do the basic building blocks, the nucleobases in the pond, come from in the first place? The chemistry of the surrounding atmosphere, dominated by carbon dioxide (CO2), nitrogen gas (N2), sulfur dioxide (SO2), and water (H2O), is of no great help. Under the conditions on early Earth ("weakly reducing atmosphere"), even the occasional bout of lightning, as in the famous Miller-Urey experiment on the origins of organic molecules, will not produce a significant amount of nucleobases.
Meteorites falling on Earth, on the other hand, are a much more plausible source. At that time, roughly 4 billion years ago, meteoritic bombardment of Earth was between 100 million and 100 billion times more intense than nowadays, with between a trillion and a quadrillion kilograms of meteoritic material raining down on Earth's surface every year, carrying an estimated 2000 kilograms per year of intact carbon compounds that have survived the cosmic journey intact. In addition, there is a steady flow of interplanetary dust particles making their way to the surface of the Earth directly, carrying along whatever surface chemicals have formed. This much less spectacular arrival is nonetheless quite effective, amounting to an estimated 60 million kilograms of intact carbon compounds per year.
Meteorites seeding warm little ponds: a quantitative study
It's all very well to talk about a scenario such as meteorites or dust particles carrying nucleobases into small ponds, but unless the model is backed by quantitative data, it does not have much more explanatory power than a Just-So Story.
Pearce and his colleagues calculated a detailed model for this scenario. From a reconstructed history of the Moon's impact craters, they derived three possible scenarios for meteoritic bombardments of the Earth – a late bombardment model, with a late onset of intense meteoritic bombardment at about 3.9 billion years before the present, plus two additional models, both setting in around 4.5 billion years before the present, and representing the minimum and maximum amount of meteoric material compatible with the data, respectively.
They then calculated the probability of sizeable carbon-containing (carbonaceous) meteorites “seeding” these ponds. Specifically, these meteorites, which are originally between about 20 and 40 meter in diameter, break up into small pieces as they traverse Earth’s atmosphere. The astronomers calculated the probability of such small pieces landing near a suitably sized warm little pond on Earth (between 1 and 10 meter in diameter), close enough for some of its organic material to enter the pond. (For this calculation, they had to estimate the number of warm little ponds; they did so by assuming a similar prevalence of ponds on landmasses as today, and accounting for the overall smaller landmass area back then, based on models of geological evolution. To be on the safe side, they repeated their calculation both for ten times as many and for one tenth as many ponds.) The result is that thousands of wet little ponds would have been seeded in this way, providing them with building blocks for emergent life.
Simulating what happens in warm little ponds
What happens to the meteorite- or dust-born nucleobases once they have entered the pond? A number of them will be lost: While the pond is filled with water, during one of the wet phases, nucleobases will be dissolved in water (hydrolysis). Some of the water will seep through pores in the basalt base of the pond, taking nucleobases with it, and removing those nucleobases from any further chemical reactions within the pond. During the dry phases, when the pond is dried up and its chemicals deposited as sediments, UV radiation from the Sun will split nucleobases into simpler compounds (photo-disssociation) – unless these nucleobases are protected by sediments on top.
With new nucleobases introduced into ponds at a certain rates, and with various mechanisms for nucleobase loss, it is quite clear that only quantitative modeling can tell whether or not there remained sufficiently many nucleobases in a number of ponds for longer chains of RNA to form. Again, the researchers considered several possibilities for dryer and wetter, hotter and colder conditions on the early Earth. These conditions will also determine how fast or slow nucleobases group together to form RNA chains.
Building block delivery: meteors, not dust grains
The first interesting result to come out of the study is that meteors, not interplanetary dust grains are the main source for nucleobases that survive the various adverse conditions. The reason is simply that the steady deposition by dust grains competes directly with the mechanisms for nucleobase loss, such as seepage and photo-dissociation. Meteorites, on the other hand, will deposit a considerable amount of nucleobases in one go, leading to higher nucleobase concentrations at least for a shorter time.
But, as it turns out, that shorter time is enough for the nucleobases to form longer RNA molecules, and those, in turn, are not lost as readily as their shorter kin. In particular, these larger molecules do not seep away through basalt pores, due to their larger size. That is how longer RNA molecules, once formed, can survive to take part in more complex chemical reactions – and why this happens on the basis of the nucleobases deposited in bulk by meteorites, but not with the steady stream of nucleobases deposited by interplanetary dust particles.
The case for quickly forming life
The deposition model has interesting implications for the timing of the origin of life. Over time, the meteorite infall rate quickly decreases, so there is a comparatively short window of opportunity. Most of the nucleobase delivery by meteorites must have occurred rather early, until about 4.17 billion years before our time. This suggests that the RNA world should have formed rather early, as well, namely 200 to 300 million years after the surface of the Earth had cooled sufficiently to become habitable – that is, after the temperatures had dropped sufficiently for areas of liquid water, such as oceans and lakes, to form on Earth surface.
Again, we are probably a few years off finding a complete, consistent, generally accepted model of how life originated on Earth. The calculation published now by Pearce and his colleagues is one piece of the puzzle – demonstrating that meteorites are likely to play a major role in bringing the building blocks of life to Earth, and that under those circumstances, longer RNA pieces would have formed comparatively early in Earth's history. Overall, the calculations increase the feasibility of the warm little pond scenario, strengthening that scenario’s position in comparison with the competing hydrothermal vent scenario.
But on the path towards a standard model, we need quantitative analyses such as the one describe here – calculations that combine our knowledge about the geology of the early Earth, chemical conditions, properties of the molecules involved, and astronomical information about the properties of meteorites and interplanetary dust, to tell us which of the hypothetical steps from simple chemicals to self-reproducing living cells are feasible and which aren't. It is an exciting feature of current research on the origins of life that thanks to advances in many fields, from microbiology to the search for exoplanets and observations of planetary birthplaces around stars, we are steadily moving away from speculations and into the realm of quantitative analyses.